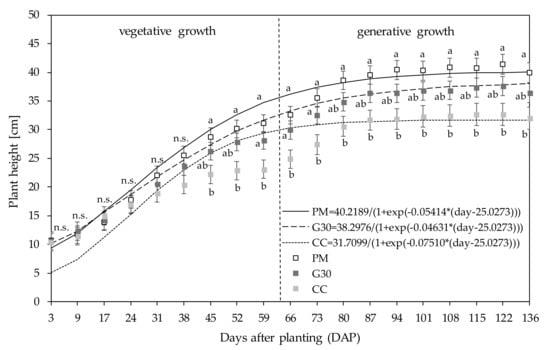
Impact of Different Growing Substrates on Growth, Yield and Cannabinoid Content of Two Cannabis sativa L. Genotypes in a Pot Culture
The impacts of different growing substrate compositions, consisting of peat (PM), peat substituted with 30% green fibre (G30) and coco coir fibre (CC) growth media, were investigated in regard to the plant height, biomass and floral yield, biomass nitrogen (N) content, root growth, and...
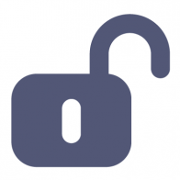
To facilitate root harvesting and processing, aeroponic (AP) and aeroponic-elicited cultures (AEP) were established and compared to soil-cultivated plants (SP). Interestingly, considerably increased plant growth—particularly of the roots—and a significant increase (up to 20-fold in the case of β-sitosterol) in the total content of the aforementioned roots’ bioactive molecules were observed in AP and AEP.
In conclusion, aeroponics, an easy, standardized, contaminant-free cultivation technique, facilitates the harvesting/processing of roots along with a greater production of their secondary bioactive metabolites, which could be utilized in the formulation of health-promoting and health-care products.
5. Conclusions
The results of this study showed that different substrate compositions, namely coco coir fibres (CC), standard peat-based media (PM) and peat substituted with 30% of green fibres (G30), had significant impacts on the growth, biomass yields, root development and nitrogen (N) tissue content of C. sativa after harvest.The use of CC as a growing media indicated a reduction in total plant height, leaf N content, leaf DW yields and root length density (RLD) compared to PM and G30 growing media.
Both phytocannabinoid-rich cannabis genotypes reacted in a genotype-specific manner on flower yields. Whereas KAN had the highest floral yield when grown in PM, 0.2x showed no significant differences, with higher yields grown in G30 and CC compared to KAN.
A limiting effect on the CBD/A content enacted by the different substrates could not be confirmed. The impact of different substrate compositions on the growth, development and cannabinoid content of C. sativa is a major issue when considering cannabis’ use as a botanical therapeutic, ideally with a fixed dosage of active compound, with a small range of variation.
It can be concluded that the use of organic green fibres to partly replace the fractionated peat showed a genotype-specific option for constant plant development, a comparable high biomass yield and a stable cannabinoid content, compared to a peat containing standard substrate.